The main research areas of the laboratory are
- Optic flow processing in the pretectum and optic tectum
- Visual circuit adaptations to natural evironments
- Functional architecture of the neural integrator for horizontal eye movements
- Development of binocular motor coordination in zebrafish larvae and juveniles
- Zebrafish eye movements
- Efficacy of suitable anaesthetic agents in zebrafish
Optic flow processing in the pretectum and optic tectum
Animals are able to perceive self-motion and navigate in their environment using optic flow information. They often perform visually guided stabilization behaviors like the optokinetic (OKR) or optomotor response (OMR) in order to maintain their eye and body position relative to the moving surround. But how does the animal manage to perform appropriate behavioral response and how are processing tasks divided between the various non-cortical visual brain areas? Experiments have shown that the zebrafish pretectum, which is homologous to the mammalian accessory optic system, is involved in the OKR and OMR. The optic tectum (superior colliculus in mammals) is involved in processing of small stimuli, e.g. during prey capture. We have previously shown that many pretectal neurons respond selectively to rotational or translational motion. These neurons are likely detectors for specific optic flow patterns and mediate behavioral choices of the animal based on optic flow information. We investigate the motion feature extraction of brain structures that receive input from retinal ganglion cells to identify the visual computations that underlie behavioral decisions during prey capture, OKR, OMR and other visually mediate behaviors. Our study of receptive fields shows that receptive field sizes in pretectum (large) and tectum (small) are very different and that pretectal responses are diverse and anatomically organized.
Since calcium indicators are slow and receptive fields for motion stimuli are difficult to measure, we also develop novel stimuli and statistical methods to infer the neuronal computations of visual brain areas.
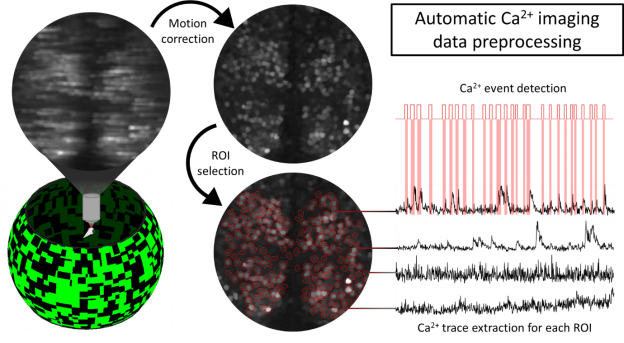
Visual circuit adaptations to natural environments
Moving gratings and dots, light chirps or noise stimuli go a long way to characterize the fundamental building blocks and important functional properties of visual systems.
When studying a sensory system it is paramount to gain basic insights on what individual components of that system respond to and how their activity is modulated by varying parameters in simplified representations of appropriate stimuli.
However, animal species have evolved within, and in conjunction with, their respective, complexly structured habitats. It is a generally accepted belief in modern neuroscience that neuronal systems are adapted to the natural statistics of the outside world in order to increase the efficiency of their computations.
We are therefore interested in how the zebrafish visual system is adapted to the zebrafish’s natural habitat and behavior.
We started investigating zebrafish-specific adaptations and plan to compare them to those of the African cichlid species A. burtoni, which naturally occupies different habitats than zebrafish and which exhibits natural behaviors and social dynamics which differ from those of zebrafish.
With this approach we expect to be able to pinpoint specific differences in behavior or habitat structure which correspond to differences in the way visual information is processed in the vertebrate visual system.
Functional architecture of the neural integrator for horizontal eye movements
To keep the eyes still and maintain a stable image on the retina seems like a very trivial thing to do, however there are several circuits involved to achieve that task. One of these circuits is the oculomotor integrator for eye positions, which transforms transient eye velocity signals into a persistent eye position signals and thereby forms a short-term memory of eye position in the hindbrain. This circuit, in humans as well as in fish, allows to keep a stable eye position in the dark (otherwise the eyes would quickly drift back to the null position after any eye movement). We aim to understand how the persistent activity is generated in this circuit and how the integrator is organized to achieve this task. Persistent activity is also found in other (including higher) brain areas and is a property of the brain that is of fundamental importance, when a delayed or prolonged response to input is needed. Investigation of mechanisms of persistent activity in the larval oculomotor hindbrain is promising, since only a relatively small number of cells contribute and the final output of the circuit (eye position) can easily be measured.
For a comprehensive understanding of oculomotor circuit computations in larval zebrafish, individual oculomotor nuclei and their functional properties need to be determined and related to the existing literature for other species. We therefore started our investigation into persistent activity mechanisms by carefully characterizing the tuning properties of hindbrain neurons to position and velocity of the left and the right eye. We identified different anatomical regions and revealed the functional architecture of the oculomotor integrator, the nucleus abducens, as well as anatomical structures encoding eye velocity (Area II and the burst system).
In the lab we, use different open- and close loop protocols to investigate the various tuning properties of these cells and to address the monocular/binocular code underlying oculomotor beheavior in larval zebrafish.
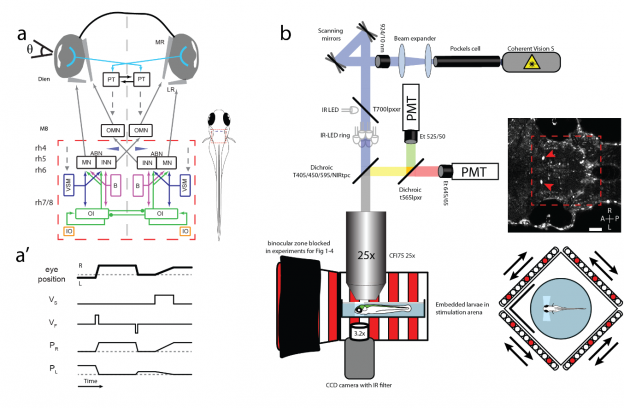
a: Circuit schematic for horizontal eye movements. Red dashed rectangle represents imaged brain area, blue cones show location of Mauthner cells. ABN: abducens nucleus; B: burst neurons; Dien: diencephalon; INN: internuclear neurons; IO: inferior olive; LR: lateral rectus; MB: midbrain; MN: motor neurons; MR: medial rectus; OMN: nucleus oculomotorius; OI: oculomotor integrator; PT: pretectum; rh 4-8: rhombomeres 4-8; VSM: velocity storage mechanism; Θ: eye position. Dashed arrows indicate direct or indirect inputs from upstream visual brain areas. a’: Simplified schematic response profiles for hindbrain oculomotor neurons during eye position changes. Dashed line represents an eye position or velocity of 0. L: left PL/R: Position coding neurons left/right, R: right; VF: fast (burst) velocity neurons; VS: slow velocity neurons. b: Schematic of microscopy setup. Agarose-embedded zebrafish larvae are visually stimulated, while eye movements are recorded from below and cellular calcium signals are recorded from above via a two-photon microscope. Setup not drawn to scale, scale bar 50 µm, red arrows show GCaMP expression in the nuclei of the Mauthner cells, which serve as a landmark (blue cones in a and in cell maps). A: anterior; L: left; P: posterior; PMT: photomultiplier tubes R: right.
Development of binocular motor coordination in zebrafish larvae and juveniles
Binocular coordination is a readily observable feature of eye movement behaviour in vertebrates. The coupling of both eyes is likely needed for a stable percept of visual information in the (binocular) visual field. Animals seemingly perform these conjugate eye movements without effort, yet many of the circuit modules underlying eye movements control exclusively one eye (e.g. the motoneurons, which connect to a single muscle). So how is binocular coordination achieved during development and learning?
We study the ontogeny of oculomotor circuits and behaviour from embryonic to juvenile developmental stages (3-29 dpf) to first characterize the system and then – hopefully – reveal the mechanisms underlying this coordination. Using a stimulus protocol that maximizes uniocular eye movements (see video), we were already able to show that many neurons in the hindbrain code uniocularly for eye position or slow phase velocity. Quick eye movements (saccades, quick phases) are driven by burst neurons, which relay the signal to the cranial nerve nuclei. While we also find monocular burst neurons in the hindbrain, most of the burst neurons show a binocular tuning already at 5 dpf.
Zebrafish Eye Movements
The simultaneous observation of zebrafish eye movements and brain activity during visual stimulation allows us to measure neuronal tuning to stimulus and motor variables during animal behavior. In almost all projects of the laboratory, such measurements are part of the experiments. We have developed a software solution for this purpose, which we have named ZebEyeTrack (see Techniques). ZebEyeTrack is written in the LabVIEW (National Instruments) environment and enables simultaneous control or recording of visual stimuli and behavioral responses during calcium imaging.
Efficacy of suitable anaesthetic agents in zebrafish
Tricaine (MS-222, TMS) is the most commonly used zebrafish anaesthetic. It is thought to act via blockade of voltage-gated sodium channels, thus preventing action potentials and neural activity. However, the relative time delays of suppressing activity in neurons (anaesthetic effect) or muscles directly (paralytic effect) are not known for zebrafish and are relevant for zebrafish welfare. Furthermore, tricaine is known to provoke an aversive reaction in zebrafish before the anaesthetic becomes effective, and the dose-response curves vary across individual zebrafish. These disadvantages raise the question of whether tricaine is truly a reliable and humane anaesthetic. In order to answer this, we investigate the effects of tricaine on neural signaling in zebrafish, specifically the timescale and magnitude of the reduction of activity in both motor and sensory brain areas. While tricaine has many disadvantages, there is no chemical anaesthetic which provides a clear alternative. For this reason, we hope to compare tricaine with different methods of anaesthesia, such as gradual cooling. For this purpose, we have built a closed-loop set up in order to test zebrafish behaviour and neural activity at defined temperatures and drug concentrations, all along under tight temporal control.